Содержание
- 2. metabolic rate. Hence, it was postulated at the beginning of the 20th century that the maximum
- 3. cardiovascular risk were shown to be positively influenced by a reduction in dietary carbohydrate consumption [66–68].
- 4. shown to extend life span in S. cerevisiae by inducing mitochondrial metabolism [127,128]. Consistently, the translational
- 5. sensitive MAP-kinase cascades and redox-sensitive transcription factors) that culminate in an overall adaptive response, represented by
- 6. Harman, D. Aging: a theory based on free radical and radiation chemistry. J. Gerontol. 11:298–300; 1956.
- 7. Forsythe, C. E.; Phinney, S. D.; Fernandez, M. L.; Quann, E. E.; Wood, R. J.; Bibus,
- 8. Thierbach, R.; Schulz, T. J.; Isken, F.; Voigt, A.; Mietzner, B.; Drewes, G.; von Kleist-Retzow, J.
- 9. diabetes: a randomized, double-blind, placebo-controlled trial. J. Hypertens. 25: 227–234; 2007. [175] Lippman, S. M.; Klein,
- 11. Скачать презентацию
Слайд 2metabolic rate. Hence, it was postulated at the beginning of the 20th
metabolic rate. Hence, it was postulated at the beginning of the 20th
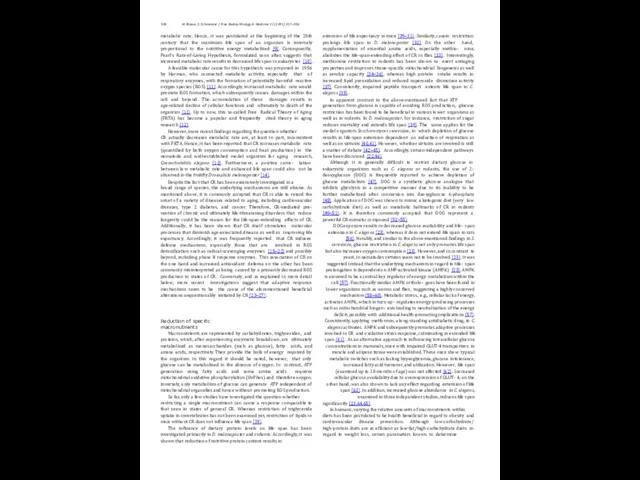
A feasible molecular cause for this hypothesis was proposed in 1956 by Harman, who connected metabolic activity, especially that of respiratory enzymes, with the formation of potentially harmful reactive oxygen species (ROS) [11]. Accordingly, increased metabolic rate would promote ROS formation, which subsequently causes damages within the cell and beyond. The accumulation of these damages results in age-related decline of cellular functions and ultimately to death of the organism [11]. Up to now, this so-called Free Radical Theory of Aging (FRTA) has become a popular and frequently cited theory in aging research [12].
However, more recent findings regarding the question whether
CR actually decreases metabolic rate are, at least in part, inconsistent with FRTA. Hence, it has been reported that CR increases metabolic rate (quantified by both oxygen consumption and heat production) in the nematode and well-established model organism for aging research, Caenorhabditis elegans [13]. Furthermore, a positive corre- lation between low metabolic rate and enhanced life span could also not be observed in the fruitfly Drosophila melanogaster [14].
Despite the fact that CR has been extensively investigated in a
broad range of species, the underlying mechanisms are still elusive. As mentioned above, it is commonly accepted that CR is able to retard the onset of a variety of diseases related to aging, including cardiovascular diseases, type 2 diabetes, and cancer. Therefore, CR-mediated pre- vention of chronic and ultimately life-threatening disorders that reduce longevity could be the reason for the life-span-extending effects of CR. Additionally, it has been shown that CR itself stimulates molecular processes that diminish age-associated disease as well as improving life expectancy. Accordingly, it was frequently reported that CR induces defense mechanisms, especially those that are involved in ROS detoxification such as radical-scavenging enzymes [15–22] and possibly beyond, including phase II response enzymes. This association of CR on the one hand and increased antioxidant defense on the other has been commonly misinterpreted as being caused by a primarily decreased ROS production in states of CR. Conversely, and as explained in more detail below, more recent investigations suggest that adaptive response mechanisms seem to be the cause of the aforementioned beneficial alterations unquestionably initiated by CR [23–27].
Reduction of specific macronutrients
Macronutrients are represented by carbohydrates, triglycerides, and proteins, which, after experiencing enzymatic breakdown, are ultimately metabolized as monosaccharides (such as glucose), fatty acids, and amino acids, respectively. They provide the bulk of energy required by the organism. In this regard it should be noted, however, that only glucose can be metabolized in the absence of oxygen. In contrast, ATP generation using fatty acids and some amino acids requires mitochondrial oxidative phosphorylation (OxPhos) and therefore oxygen. Inversely, only metabolism of glucose can generate ATP independent of mitochondrial organelles and hence without promoting ROS production.
So far, only a few studies have investigated the question whether
restricting a single macronutrient can cause a response comparable to that seen in states of general CR. Whereas restriction of triglyceride uptake in invertebrates has not been examined yet, restriction of lipids in mice without CR does not influence life span [28].
The influence of dietary protein levels on life span has been investigated primarily in D. melanogaster and rodents. Accordingly, it was shown that reduction of nutritive protein content results in
extension of life expectancy in mice [29–31]. Similarly, casein restriction prolongs life span in D. melanogaster [32]. On the other hand, supplementation of essential amino acids, especially methio- nine, abolishes the life-span-extending effect of CR in flies [33]. Interestingly, methionine restriction in rodents has been shown to exert antiaging properties and improves tissue-specific mitochondrial biogenesis as well as aerobic capacity [34–36], whereas high protein intake results in increased lipid peroxidation and reduced superoxide dismutase activity [37]. Consistently, impaired peptide transport extents life span in C. elegans [38].
In apparent contrast to the above-mentioned fact that ATP
generation from glucose is capable of avoiding ROS production, glucose restriction has been found to be beneficial in various lower organisms as well as in rodents. In D. melanogaster, for instance, restriction of sugar reduces mortality and extends life span [39]. The same applies for the model organism Saccharomyces cerevisiae, in which depletion of glucose results in life-span extension dependent on induction of respiration as well as on sirtuins [40,41]. However, whether sirtuins are involved is still a matter of debate [42–45]. Accordingly, sirtuin-independent pathways have been discussed [22,46].
Although it is generally difficult to restrict dietary glucose in eukaryotic organisms such as C. elegans or rodents, the use of 2- deoxyglucose (DOG) is frequently reported to achieve depletion of glucose metabolism [47]. DOG is a synthetic glucose analogue that inhibits glycolysis in a competitive manner due to its inability to be further metabolized after conversion into deoxyglucose 6-phosphate [48]. Application of DOG was shown to mimic a ketogenic diet (very low carbohydrate diet) as well as metabolic hallmarks of CR in rodents [49–51]. It is therefore commonly accepted that DOG represent a powerful CR-mimetic compound [52–55].
DOG exposure results in decreased glucose availability and life- span extension in C. elegans [23], whereas it does not extend life span in rats [56]. Notably, and similar to the above-mentioned findings in S. cerevisiae, glucose restriction in C. elegans not only promotes life span but also increases oxygen consumption [23]. However, and in contrast to yeast, in nematodes sirtuins seem not to be involved [23]. It was suggested instead that the underlying mechanism in regard to life- span prolongation is dependent on AMP-activated kinase (AMPK) [23]. AMPK is assumed to be a central key regulator of energy metabolism within the cell [57]. Functionally similar AMPK ortholo- gues have been found in lower organisms such as worms and flies, suggesting a highly conserved mechanism [58–60]. Metabolic stress, e.g., cellular lack of energy, activates AMPK, which in turn up- regulates energy-producing processes such as mitochondrial biogen- esis leading to neutralization of the energy deficit, possibly with additional health-promoting implications [57]. Consistently, applying metformin, a long-standing antidiabetic drug, to C. elegans activates AMPK and subsequently promotes adaptive processes involved in CR and oxidative stress response, culminating in extended life span [61]. As an alternative approach to influencing intracellular glucose concentrations in mammals, mice with impaired GLUT-4 transporters in muscle and adipose tissue were established. These mice show typical metabolic switches such as fasting hyperglycemia, glucose intolerance, increased fatty acid turnover, and utilization. However, life span (examined up to 18 months of age) was not affected [62]. Increased cellular glucose availability due to overexpression of GLUT- 4, on the other hand, was also shown to lack any effect regarding extension of life span [63]. In addition, increased glucose abundance in C. elegans, examined in three independent studies, reduces life span
significantly [23,64,65].
In humans, varying the relative amounts of macronutrients within
diets has been postulated to be health beneficial in regard to obesity and cardiovascular disease prevention. Although low-carbohydrate/ high-protein diets are as efficient as low-fat/high-carbohydrate diets in regard to weight loss, serum parameters known to determine
328 M. Ristow, S. Schmeisser / Free Radical Biology & Medicine 51 (2011) 327–336
Слайд 3cardiovascular risk were shown to be positively influenced by a reduction in
cardiovascular risk were shown to be positively influenced by a reduction in
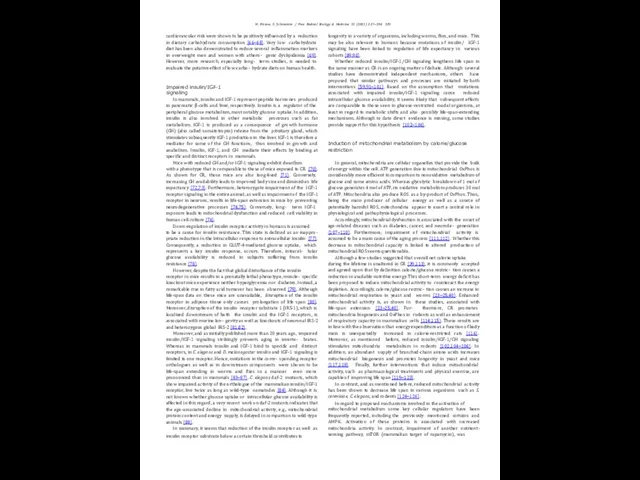
Impaired insulin/IGF-1 signaling
In mammals, insulin and IGF-1 represent peptide hormones produced in pancreatic β-cells and liver, respectively. Insulin is a regulator of the peripheral glucose metabolism, most notably glucose uptake. In addition, insulin is also involved in other metabolic processes such as fat metabolism. IGF-1 is produced as a consequence of growth hormone (GH) (also called somatotropin) release from the pituitary gland, which stimulates subsequently IGF-1 production in the liver. IGF-1 is therefore a mediator for some of the GH functions, thus involved in growth and anabolism. Insulin, IGF-1, and GH mediate their effects by binding at specific and distinct receptors in mammals.
Mice with reduced GH and/or IGF-1 signaling exhibit dwarfism
with a phenotype that is comparable to those of mice exposed to CR [70]. As shown for CR, those mice are also long-lived [71]. Conversely, increasing GH availability leads to improved body size and diminishes life expectancy [72,73]. Furthermore, heterozygote impairment of the IGF-1 receptor signaling in the entire animal, as well as impairment of the IGF-1 receptor in neurons, results in life-span extension in mice by preventing neurodegenerative processes [74,75]. Conversely, long- term IGF-1 exposure leads to mitochondrial dysfunction and reduced cell viability in human cell culture [76].
Down-regulation of insulin receptor activity in humans is assumed
to be a cause for insulin resistance. This state is defined as an inappro- priate reduction in the intracellular response to extracellular insulin [77]. Consequently, a reduction in GLUT-4-mediated glucose uptake, which represents a key insulin response, occurs. Therefore, intracel- lular glucose availability is reduced in subjects suffering from insulin resistance [78].
However, despite the fact that global disturbance of the insulin
receptor in mice results in a prenatally lethal phenotype, muscle- specific knockout mice experience neither hyperglycemia nor diabetes. Instead, a remarkable rise in fatty acid turnover has been observed [79]. Although life-span data on these mice are unavailable, disruption of the insulin receptor in adipose tissue only causes prolongation of life span [80]. Moreover, disruption of the insulin receptor substrate 1 (IRS-1), which is localized downstream of both the insulin and the IGF-1 receptors, is associated with murine lon- gevity as well as knockouts of neuronal IRS-2 and heterozygous global IRS-2 [81,82].
Moreover, and as initially published more than 20 years ago, impaired insulin/IGF-1 signaling strikingly prevents aging in inverte- brates. Whereas in mammals insulin and IGF-1 bind to specific and distinct receptors, in C. elegans and D. melanogaster insulin and IGF-1 signaling is limited to one receptor. Hence, mutations in the corre- sponding receptor orthologues as well as in downstream components were shown to be life-span extending in worms and flies in a manner even more pronounced than in mammals [83–87]. C. elegans daf-2 mutants, which show impaired activity of the orthologue of the mammalian insulin/IGF-1 receptor, live twice as long as wild-type nematodes [84]. Although it is not known whether glucose uptake or intracellular glucose availability is affected in this regard, a very recent work on daf-2 mutants indicates that the age-associated decline in mitochondrial activity, e.g., mitochondrial protein content and energy supply, is delayed in comparison to wild-type animals [88].
In summary, it seems that reduction of the insulin receptor as well as insulin receptor substrate below a certain threshold contributes to
longevity in a variety of organisms, including worms, flies, and mice. This may be also relevant to humans because mutations of insulin/ IGF-1 signaling have been linked to regulation of life expectancy in various cohorts [89,90].
Whether reduced insulin/IGF-1/GH signaling lengthens life span in the same manner as CR is an ongoing matter of debate. Although several studies have demonstrated independent mechanisms, others have proposed that similar pathways and processes are initiated by both interventions [59,91–101]. Based on the assumption that mutations associated with impaired insulin/IGF-1 signaling cause reduced intracellular glucose availability, it seems likely that subsequent effects are comparable to those seen in glucose-restricted model organisms, at least in regard to metabolic shifts and also possibly life-span-extending mechanisms. Although to date direct evidence is missing, some studies provide support for this hypothesis [102–106].
Induction of mitochondrial metabolism by calorie/glucose restriction
In general, mitochondria are cellular organelles that provide the bulk of energy within the cell. ATP generation due to mitochondrial OxPhos is considerably more efficient in comparison to nonoxidative metabolism of glucose and some amino acids. Whereas glycolytic breakdown of 1 mol of glucose generates 4 mol of ATP, its oxidative metabolism produces 30 mol of ATP. Mitochondria also produce ROS as a by-product of OxPhos. Thus, being the main producer of cellular energy as well as a source of potentially harmful ROS, mitochondria appear to exert a central role in physiological and pathophysiological processes.
Accordingly, mitochondrial dysfunction is associated with the onset of age-related diseases such as diabetes, cancer, and neurode- generation [107–110]. Furthermore, impairment of mitochondrial activity is assumed to be a main cause of the aging process [111,112]. Whether this decrease in mitochondrial capacity is linked to altered production of mitochondrial ROS seems questionable.
Although a few studies suggested that overall net calorie uptake
during the lifetime is unaltered in CR [39,113], it is commonly accepted and agreed upon that by definition calorie/glucose restric- tion causes a reduction in available nutritive energy. This short-term energy deficit has been proposed to induce mitochondrial activity to counteract the energy depletion. Accordingly, calorie/glucose restric- tion causes an increase in mitochondrial respiration in yeast and worms [23–25,40]. Enhanced mitochondrial activity is, as shown in these studies, associated with life-span extension [23–25,40]. Fur- thermore, CR promotes mitochondria biogenesis and OxPhos in rodents as well as enhancement of respiratory capacity in mammalian cells [114,115]. These results are in line with the observation that energy expenditure as a function of body mass is unexpectedly increased in calorie-restricted rats [116]. Moreover, as mentioned before, reduced insulin/IGF-1/GH signaling stimulates mitochondria metabolism in rodents [102,104–106]. In addition, an abundant supply of branched-chain amino acids increases mitochondrial biogenesis and promotes longevity in yeast and mice [117,118]. Finally, further interventions that induce mitochondrial activity, such as pharmacological treatments and physical exercise, are capable of improving life span [119–123].
In contrast, and as mentioned before, reduced mitochondrial activity has been shown to decrease life span in various organisms such as S. cerevisiae, C. elegans, and rodents [124–126].
In regard to proposed mechanisms involved in the activation of
mitochondrial metabolism some key cellular regulators have been frequently reported, including the previously mentioned sirtuins and AMPK. Activation of these proteins is associated with increased mitochondria activity. In contrast, impairment of another nutrient- sensing pathway, mTOR (mammalian target of rapamycin), was
M. Ristow, S. Schmeisser / Free Radical Biology & Medicine 51 (2011) 327–336 329
Слайд 4shown to extend life span in S. cerevisiae by inducing mitochondrial metabolism
shown to extend life span in S. cerevisiae by inducing mitochondrial metabolism
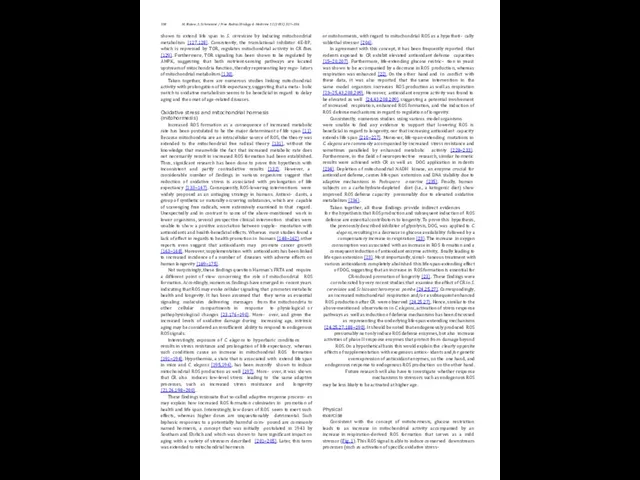
Taken together, there are numerous studies linking mitochondrial activity with prolongation of life expectancy, suggesting that a meta- bolic switch to oxidative metabolism seems to be beneficial in regard to delay aging and the onset of age-related diseases.
Oxidative stress and mitochondrial hormesis (mitohormesis)
Increased ROS formation as a consequence of increased metabolic rate has been postulated to be the major determinant of life span [11]. Because mitochondria are an intracellular source of ROS, the theory was extended to the mitochondrial free radical theory [131], without the knowledge that meanwhile the fact that increased metabolic rate does not necessarily result in increased ROS formation had been established. Thus, significant research has been done to prove this hypothesis with inconsistent and partly contradictive results [132]. However, a considerable number of findings in various organisms suggest that reduction of oxidative stress is associated with prolongation of life expectancy [133–147]. Consequently, ROS-lowering interventions were widely proposed as an antiaging strategy in humans. Antioxi- dants, a group of synthetic or naturally occurring substances, which are capable of scavenging free radicals, were extensively examined in that regard. Unexpectedly and in contrast to some of the above-mentioned work in lower organisms, several prospective clinical intervention studies were unable to show a positive association between supple- mentation with antioxidants and health-beneficial effects. Whereas most studies found a lack of effect in regards to health promotion in humans [148–162], other reports even suggest that antioxidants may promote cancer growth [163–168]. Moreover, supplementation with antioxidants has been linked to increased incidence of a number of diseases with adverse effects on human longevity [169–175].
Not surprisingly, these findings question Harman's FRTA and require a different point of view concerning the role of mitochondrial ROS formation. Accordingly, numerous findings have emerged in recent years indicating that ROS may evoke cellular signaling that promotes metabolic health and longevity. It has been assumed that they serve as essential signaling molecules delivering messages from the mitochondria to other cellular compartments in response to physiological or pathophysiological changes [23,176–190]. More- over, and given the increased levels of oxidative damage during increasing age, intrinsic aging may be considered an insufficient ability to respond to endogenous ROS signals.
Interestingly, exposure of C. elegans to hyperbaric conditions
results in stress resistance and prolongation of life expectancy, whereas such conditions cause an increase in mitochondrial ROS formation [191–194]. Hypothermia, a state that is associated with extend life span in mice and C. elegans [195,196], has been recently shown to induce mitochondrial ROS production as well [197]. More- over, it was shown that CR also induces low-level stress leading to the same adaptive processes, such as increased stress resistance and longevity [21,26,198–200].
These findings insinuate that so-called adaptive response process- es may explain how increased ROS formation culminates in promotion of health and life span. Interestingly, low doses of ROS seem to exert such effects, whereas higher doses are unquestionably detrimental. Such biphasic responses to a potentially harmful com- pound are commonly named hormesis, a concept that was initially postulated in 1943 by Southam and Ehrlich and which was shown to have significant impact on aging with a variety of stressors described [201–205]. Later, this term was extended to mitochondrial hormesis
or mitohormesis, with regard to mitochondrial ROS as a hypotheti- cally sublethal stressor [206].
In agreement with this concept, it has been frequently reported that rodents exposed to CR exhibit elevated antioxidant defense capacities [15–20,207]. Furthermore, life-extending glucose restric- tion in yeast was shown to be accompanied by a decrease in ROS production, whereas respiration was enhanced [22]. On the other hand and in conflict with these data, it was also reported that the same intervention in the same model organism increases ROS production as well as respiration [23–25,43,208,209]. Moreover, antioxidant enzyme activity was found to be elevated as well [24,43,208,209], suggesting a potential involvement of increased respiration, enhanced ROS formation, and the induction of ROS defense mechanisms in regard to regulation of longevity.
Consistently, numerous studies using various model organisms
were unable to find any evidence to support that lowering ROS is beneficial in regard to longevity, nor that increasing antioxidant capacity extends life span [210–227]. Moreover, life-span-extending mutations in C. elegans are commonly accompanied by increased stress resistance and sometimes paralleled by enhanced metabolic activity [228–233]. Furthermore, in the field of neuroprotective research, similar hormetic results were achieved with CR as well as DOG application in rodents [234]. Depletion of mitochondrial NADH kinase, an enzyme crucial for antioxidant defense, causes life-span extension and DNA stability due to adaptive mechanisms in Podospora anserine [235]. Finally, human subjects on a carbohydrate-depleted diet (i.e., a ketogenic diet) show improved ROS defense capacity presumably due to elevated oxidative metabolism [236].
Taken together, all these findings provide indirect evidences
for the hypothesis that ROS production and subsequent induction of ROS defense are essential contributors to longevity. To prove this hypothesis, the previously described inhibitor of glycolysis, DOG, was applied to C. elegans, resulting in a decrease in glucose availability followed by a compensatory increase in respiration [23]. The increase in oxygen consumption was associated with an increase in ROS formation and a consequent induction of antioxidant enzyme activity, finally leading to life-span extension [23]. Most importantly, simul- taneous treatment with various antioxidants completely abolished this life-span-extending effect of DOG, suggesting that an increase in ROS formation is essential for CR-induced promotion of longevity [23]. These findings were corroborated by very recent studies that examine the effect of CR in S. cerevisiae and Schizosaccharomyces pombe [24,25,27]. Correspondingly, an increased mitochondrial respiration and/or a subsequent enhanced ROS production after CR were observed [24,25,27]. Hence, similar to the above-mentioned observations in C. elegans, activation of stress response pathways as well as induction of defense mechanisms has been discussed as representing the underlying life-span-extending mechanisms [24,25,27,188–190]. It should be noted that endogenously produced ROS presumably not only induce ROS defense enzymes, but also increase activities of phase II response enzymes that protect from damage beyond ROS. On a hypothetical basis this would explain the clearly opposite effects of supplementation with exogenous antiox- idants and/or genetic overexpression of antioxidant enzymes, on the one hand, and endogenous response to endogenous ROS production on the other hand. Future research will also have to investigate whether response mechanisms to stressors such as endogenous ROS
may be less likely to be activated at higher age.
Physical exercise
Consistent with the concept of mitohormesis, glucose restriction leads to an increase in mitochondrial activity accompanied by an increase in respiration-derived ROS formation that serves as a mild stressor (Fig. 1). This ROS signal is able to induce conserved downstream processes (such as activation of specific oxidative stress-
330 M. Ristow, S. Schmeisser / Free Radical Biology & Medicine 51 (2011) 327–336
Слайд 5sensitive MAP-kinase cascades and redox-sensitive transcription factors) that culminate in an overall
sensitive MAP-kinase cascades and redox-sensitive transcription factors) that culminate in an overall
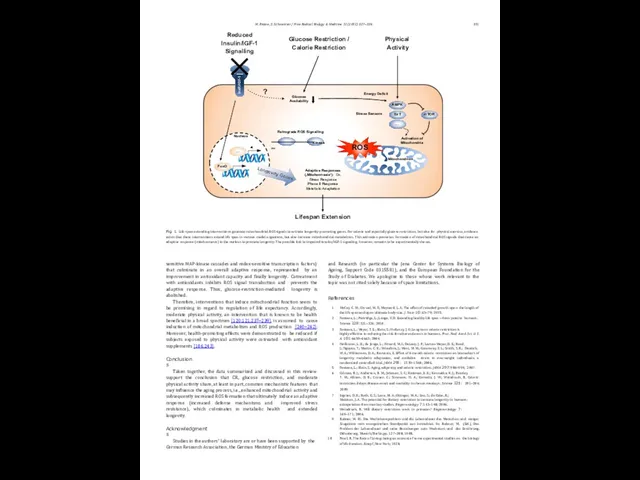
Therefore, interventions that induce mitochondrial function seem to be promising in regard to regulation of life expectancy. Accordingly, moderate physical activity, an intervention that is known to be health beneficial in a broad spectrum [120,121,237–239], is assumed to cause induction of mitochondrial metabolism and ROS production [240–242]. Moreover, health-promoting effects were demonstrated to be reduced if subjects exposed to physical activity were cotreated with antioxidant supplements [186,243].
Conclusions
Taken together, the data summarized and discussed in this review support the conclusion that CR, glucose restriction, and moderate physical activity share, at least in part, common mechanistic features that may influence the aging process, i.e., enhanced mitochondrial activity and subsequently increased ROS formation that ultimately induce an adaptive response (increased defense mechanisms and improved stress resistance), which culminates in metabolic health and extended longevity.
Acknowledgments
Studies in the authors’ laboratory are or have been supported by the German Research Association, the German Ministry of Education
and Research (in particular the Jena Center for Systems Biology of Ageing, Support Code 0315581), and the European Foundation for the Study of Diabetes. We apologize to those whose work relevant to the topic was not cited solely because of space limitations.
References
McCay, C. M.; Crowel, M. F.; Maynard, L. A. The effect of retarded growth upon the length of the life span and upon ultimate body size. J. Nutr. 10:63–79; 1935.
Fontana, L.; Partridge, L.; Longo, V. D. Extending healthy life span—from yeast to humans. Science 328:321–326; 2010.
Fontana, L.; Meyer, T. E.; Klein, S.; Holloszy, J. O. Long-term calorie restriction is
highly effective in reducing the risk for atherosclerosis in humans. Proc. Natl Acad. Sci. U. S. A. 101:6659–6663; 2004.
Heilbronn, L. K.; de Jonge, L.; Frisard, M. I.; DeLany, J. P.; Larson-Meyer, D. E.; Rood,
J.; Nguyen, T.; Martin, C. K.; Volaufova, J.; Most, M. M.; Greenway, F. L.; Smith, S. R.; Deutsch, W. A.; Williamson, D. A.; Ravussin, E. Effect of 6-month calorie restriction on biomarkers of longevity, metabolic adaptation, and oxidative stress in overweight individuals: a randomized controlled trial. JAMA 295: 1539–1548; 2006.
Fontana, L.; Klein, S. Aging, adiposity, and calorie restriction. JAMA 297:986–994; 2007.
Colman, R. J.; Anderson, R. M.; Johnson, S. C.; Kastman, E. K.; Kosmatka, K. J.; Beasley,
T. M.; Allison, D. B.; Cruzen, C.; Simmons, H. A.; Kemnitz, J. W.; Weindruch, R. Caloric restriction delays disease onset and mortality in rhesus monkeys. Science 325: 201–204; 2009.
Ingram, D. K.; Roth, G. S.; Lane, M. A.; Ottinger, M. A.; Zou, S.; de Cabo, R.;
Mattison, J. A. The potential for dietary restriction to increase longevity in humans: extrapolation from monkey studies. Biogerontology 7:143–148; 2006.
Weindruch, R. Will dietary restriction work in primates? Biogerontology 7:
169–171; 2006.
Rubner, M. III. Das Wachstumsproblem und die Lebensdauer des Menschen und einiger Säugetiere vom energetischen Standpunkt aus betrachtet. In: Rubner, M. (Ed.), Das Problem der Lebensdauer und seine Beziehungen zum Wachstum und der Ernährung. Oldenbourg, Munich/Berlin,pp. 127–208; 1908.
Pearl, R. The Rate of Living: being an account of some experimental studies on the biology of life duration. Knopf, New York; 1928.
InsR/IGF-1
Physical Activity
Reduced Insulin/IGF-1 Signalling
Glucose Restriction / Calorie Restriction
Adaptive Responses (‚Mitohormesis‘): Ox. Stress Response Phase II Response Metabolic Adaptation
Lifespan Extension
Retrograde ROS Signalling
Kinase …
Stress Sensors
Activation of Mitochondria
ROS
Energy Deficit
?
Nucleus
mTOR
SirT
…
Glucose Availability
Mitochondrion
FoxO
…
AMPK
Fig. 1. Life-span-extending interventions generate mitochondrial ROS signals to activate longevity-promoting genes. For calorie and especially glucose restriction, but also for physical exercise, evidence exists that these interventions extend life span in various model organisms, but also increase mitochondrial metabolism. This activation promotes formation of mitochondrial ROS signals that cause an adaptive response (mitohormesis) in the nucleus to promote longevity. The possible link to impaired insulin/IGF-1 signaling, however, remains to be experimentally shown.
331
M. Ristow, S. Schmeisser / Free Radical Biology & Medicine 51 (2011) 327–336
Слайд 6Harman, D. Aging: a theory based on free radical and radiation chemistry.
Harman, D. Aging: a theory based on free radical and radiation chemistry.
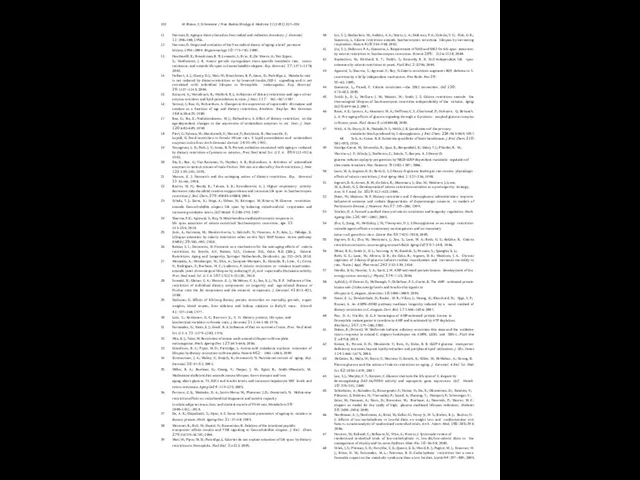
Harman, D. Origin and evolution of the free radical theory of aging: a brief personal history, 1954–2009. Biogerontology 10:773–781; 2009.
Houthoofd, K.; Braeckman, B. P.; Lenaerts, I.; Brys, K.; De Vreese, A.; Van Eygen,
S.; Vanfleteren, J. R. Axenic growth up-regulates mass-specific metabolic rate, stress resistance, and extends life span in Caenorhabditis elegans. Exp. Gerontol. 37:1371–1378; 2002.
Hulbert, A. J.; Clancy, D. J.; Mair, W.; Braeckman, B. P.; Gems, D.; Partridge, L. Metabolic rate is not reduced by dietary-restriction or by lowered insulin/IGF-1 signalling and is not correlated with individual lifespan in Drosophila melanogaster. Exp. Gerontol. 39:1137–1143; 2004.
Koizumi, A.; Weindruch, R.; Walford, R. L. Influences of dietary restriction and age on liver enzyme activities and lipid peroxidation in mice. J. Nutr. 117: 361–367; 1987.
Semsei, I.; Rao, G.; Richardson, A. Changes in the expression of superoxide dismutase and catalase as a function of age and dietary restriction. Biochem. Biophys. Res. Commun. 164:620–625; 1989.
Rao, G.; Xia, E.; Nadakavukaren, M. J.; Richardson, A. Effect of dietary restriction on the age-dependent changes in the expression of antioxidant enzymes in rat liver. J. Nutr. 120:602–609; 1990.
Pieri, C.; Falasca, M.; Marcheselli, F.; Moroni, F.; Recchioni, R.; Marmocchi, F.;
Lupidi, G. Food restriction in female Wistar rats. V. Lipid peroxidation and antioxidant enzymes in the liver. Arch. Gerontol. Geriatr. 14:93–99; 1992.
Youngman, L. D.; Park, J. Y.; Ames, B. N. Protein oxidation associated with aging is reduced by dietary restriction of protein or calories. Proc. Natl Acad. Sci. U. S. A. 89:9112–9116; 1992.
Xia, E.; Rao, G.; Van Remmen, H.; Heydari, A. R.; Richardson, A. Activities of antioxidant enzymes in various tissues of male Fischer 344 rats are altered by food restriction. J. Nutr. 125:195–201; 1995.
Masoro, E. J. Hormesis and the antiaging action of dietary restriction. Exp. Gerontol. 33:61–66; 1998.
Barros, M. H.; Bandy, B.; Tahara, E. B.; Kowaltowski, A. J. Higher respiratory activity decreases mitochondrial reactive oxygen release and increases life span in Saccharomyces cerevisiae. J. Biol. Chem. 279:49883–49888; 2004.
Schulz, T. J.; Zarse, K.; Voigt, A.; Urban, N.; Birringer, M.; Ristow, M. Glucose restriction extends Caenorhabditis elegans life span by inducing mitochondrial respiration and increasing oxidative stress. Cell Metab. 6:280–293; 2007.
Sharma, P. K.; Agrawal, V.; Roy, N. Mitochondria-mediated hormetic response in
life span extension of calorie-restricted Saccharomyces cerevisiae. Age 33:
143–154; 2010.
Zuin, A.; Carmona, M.; Morales-Ivorra, I.; Gabrielli, N.; Vivancos, A. P.; Ayte, J.; Hidalgo, E. Lifespan extension by calorie restriction relies on the Sty1 MAP kinase stress pathway. EMBO J. 29:981–991; 2010.
Rattan, S. I.; Demirovic, D. Hormesis as a mechanism for the anti-aging effects of calorie restriction. In: Everitt, A.V., Rattan, S.I.S., Couteur, D.G., Cabo, R.D. (Eds.), Calorie Restriction, Aging and Longevity. Springer Netherlands, Dordrecht, pp. 233–245; 2010.
Mesquita, A.; Weinberger, M.; Silva, A.; Sampaio-Marques, B.; Almeida, B.; Leao, C.; Costa, V.; Rodrigues, F.; Burhans, W. C.; Ludovico, P. Caloric restriction or catalase inactivation extends yeast chronological lifespan by inducing H2O2 and superoxide dismutase activity. Proc. Natl Acad. Sci. U. S. A. 107:15123–15128; 2010.
Iwasaki, K.; Gleiser, C. A.; Masoro, E. J.; McMahan, C. A.; Seo, E. J.; Yu, B. P. Influence of the restriction of individual dietary components on longevity and age-related disease of Fischer rats: the fat component and the mineral component. J. Gerontol. 43:B13–B21; 1988.
Stoltzner, G. Effects of life-long dietary protein restriction on mortality, growth, organ weights, blood counts, liver aldolase and kidney catalase in Balb/C mice. Growth 41:337–348; 1977.
Leto, S.; Kokkonen, G. C.; Barrows Jr., C. H. Dietary protein, life-span, and
biochemical variables in female mice. J. Gerontol. 31:144–148; 1976.
Fernandes, G.; Yunis, E. J.; Good, R. A. Influence of diet on survival of mice. Proc. Natl Acad. Sci. U. S. A. 73:1279–1283; 1976.
Min, K. J.; Tatar, M. Restriction of amino acids extends lifespan in Drosophila
melanogaster. Mech. Ageing Dev. 127:643–646; 2006.
Grandison, R. C.; Piper, M. D.; Partridge, L. Amino-acid imbalance explains extension of lifespan by dietary restriction in Drosophila. Nature 462: 1061–1064; 2009.
Zimmerman, J. A.; Malloy, V.; Krajcik, R.; Orentreich, N. Nutritional control of aging. Exp. Gerontol. 38:47–52; 2003.
Miller, R. A.; Buehner, G.; Chang, Y.; Harper, J. M.; Sigler, R.; Smith-Wheelock, M. Methionine-deficient diet extends mouse lifespan, slows immune and lens
aging, alters glucose, T4, IGF-I and insulin levels, and increases hepatocyte MIF levels and stress resistance. Aging Cell 4:119–125; 2005.
Perrone, C. E.; Mattocks, D. A.; Jarvis-Morar, M.; Plummer, J. D.; Orentreich, N. Methionine restriction effects on mitochondrial biogenesis and aerobic capacity
in white adipose tissue, liver, and skeletal muscle of F344 rats. Metabolism 59:
1000–1011; 2010.
De, A. K.; Chipalkatti, S.; Aiyar, A. S. Some biochemical parameters of ageing in relation to dietary protein. Mech. Ageing Dev. 21:37–48; 1983.
Meissner, B.; Boll, M.; Daniel, H.; Baumeister, R. Deletion of the intestinal peptide
transporter affects insulin and TOR signaling in Caenorhabditis elegans. J. Biol. Chem. 279:36739–36745; 2004.
Mair, W.; Piper, M. D.; Partridge, L. Calories do not explain extension of life span by dietary restriction in Drosophila. PLoS Biol. 3:e223; 2005.
Lin, S. J.; Kaeberlein, M.; Andalis, A. A.; Sturtz, L. A.; Defossez, P. A.; Culotta, V. C.; Fink, G. R.; Guarente, L. Calorie restriction extends Saccharomyces cerevisiae lifespan by increasing respiration. Nature 418:344–348; 2002.
Lin, S. J.; Defossez, P. A.; Guarente, L. Requirement of NAD and SIR2 for life-span extension by calorie restriction in Saccharomyces cerevisiae. Science 289: 2126–2128; 2000.
Kaeberlein, M.; Kirkland, K. T.; Fields, S.; Kennedy, B. K. Sir2-independent life span extension by calorie restriction in yeast. PLoS Biol. 2:E296; 2004.
Agarwal, S.; Sharma, S.; Agrawal, V.; Roy, N. Caloric restriction augments ROS defense in S. cerevisiae by a Sir2p independent mechanism. Free Radic. Res. 39:
55–62; 2005.
Guarente, L.; Picard, F. Calorie restriction—the SIR2 connection. Cell 120:
473–482; 2005.
Smith Jr., D. L.; McClure, J. M.; Matecic, M.; Smith, J. S. Calorie restriction extends the chronological lifespan of Saccharomyces cerevisiae independently of the sirtuins. Aging Cell 6:649–662; 2007.
Roux, A. E.; Leroux, A.; Alaamery, M. A.; Hoffman, C. S.; Chartrand, P.; Ferbeyre, G.; Rokeach, L. A. Pro-aging effects of glucose signaling through a G protein- coupled glucose receptor in fission yeast. PLoS Genet. 5:e1000408; 2009.
Wick, A. N.; Drury, D. R.; Nakada, H. I.; Wolfe, J. B. Localization of the primary
metabolic block produced by 2-deoxyglucose. J. Biol. Chem. 224:963–969; 1957.
Sols, A.; Crane, R. K. Substrate specificity of brain hexokinase. J. Biol. Chem. 210:
581–595; 1954.
Garriga-Canut, M.; Schoenike, B.; Qazi, R.; Bergendahl, K.; Daley, T. J.; Pfender, R. M.; Morrison, J. F.; Ockuly, J.; Stafstrom, C.; Sutula, T.; Roopra, A. 2-Deoxy-D-
glucose reduces epilepsy progression by NRSF-CtBP-dependent metabolic regulation of chromatin structure. Nat. Neurosci. 9:1382–1387; 2006.
Lane, M. A.; Ingram, D. K.; Roth, G. S. 2-Deoxy-D-glucose feeding in rats mimics physiologic effects of calorie restriction. J. Anti Aging Med. 1:327–336; 1998.
Ingram, D. K.; Anson, R. M.; de Cabo, R.; Mamczarz, J.; Zhu, M.; Mattison, J.; Lane,
M. A.; Roth, G. S. Development of calorie restriction mimetics as a prolongevity strategy. Ann. N. Y. Acad. Sci. 1019:412–423; 2004.
Duan, W.; Mattson, M. P. Dietary restriction and 2-deoxyglucose administration improve behavioral outcome and reduce degeneration of dopaminergic neurons in models of Parkinson's disease. J. Neurosci. Res. 57:195–206; 1999.
Sinclair, D. A. Toward a unified theory of caloric restriction and longevity regulation. Mech. Ageing Dev. 126:987–1002; 2005.
Zhu, Z.; Jiang, W.; McGinley, J. N.; Thompson, H. J. 2-Deoxyglucose as an energy restriction mimetic agent: effects on mammary carcinogenesis and on mammary
tumor cell growth in vitro. Cancer Res. 65:7023–7030; 2005.
Ingram, D. K.; Zhu, M.; Mamczarz, J.; Zou, S.; Lane, M. A.; Roth, G. S.; deCabo, R. Calorie restriction mimetics: an emerging research field. Aging Cell 5:97–108; 2006.
Minor, R. K.; Smith Jr., D. L.; Sossong, A. M.; Kaushik, S.; Poosala, S.; Spangler, E. L.;
Roth, G. S.; Lane, M.; Allison, D. B.; de Cabo, R.; Ingram, D. K.; Mattison, J. A. Chronic ingestion of 2-deoxy-D-glucose induces cardiac vacuolization and increases mortality in rats. Toxicol. Appl. Pharmacol. 243:332–339; 2010.
Hardie, D. G.; Hawley, S. A.; Scott, J. W. AMP-activated protein kinase: development of the energy sensor concept. J. Physiol. 574:7–15; 2006.
Apfeld, J.; O'Connor, G.; McDonagh, T.; DiStefano, P. S.; Curtis, R. The AMP- activated protein kinase aak-2 links energy levels and insulin-like signals to
lifespan in C. elegans. Genes Dev. 18:3004–3009; 2004.
Greer, E. L.; Dowlatshahi, D.; Banko, M. R.; Villen, J.; Hoang, K.; Blanchard, D.; Gygi, S. P.; Brunet, A. An AMPK–FOXO pathway mediates longevity induced by a novel method of dietary restriction in C. elegans. Curr. Biol. 17:1646–1656; 2007.
Pan, D. A.; Hardie, D. G. A homologue of AMP-activated protein kinase in
Drosophila melanogaster is sensitive to AMP and is activated by ATP depletion.
Biochem. J. 367:179–186; 2002.
Onken, B.; Driscoll, M. Metformin induces a dietary restriction-like state and the oxidative stress response to extend C. elegans healthspan via AMPK, LKB1, and SKN-1. PLoS One 5:e8758; 2010.
Kotani, K.; Peroni, O. D.; Minokoshi, Y.; Boss, O.; Kahn, B. B. GLUT4 glucose transporter deficiency increases hepatic lipid production and peripheral lipid utilization. J. Clin. Invest. 114:1666–1675; 2004.
McCarter, R.; Mejia, W.; Ikeno, Y.; Monnier, V.; Kewitt, K.; Gibbs, M.; McMahan, A.; Strong, R. Plasma glucose and the action of calorie restriction on aging. J. Gerontol. A Biol. Sci. Med. Sci. 62:1059–1070; 2007.
Lee, S. J.; Murphy, C. T.; Kenyon, C. Glucose shortens the life span of C. elegans by
downregulating DAF-16/FOXO activity and aquaporin gene expression. Cell Metab. 10:379–391; 2009.
Schlotterer, A.; Kukudov, G.; Bozorgmehr, F.; Hutter, H.; Du, X.; Oikonomou, D.; Ibrahim, Y.; Pfisterer, F.; Rabbani, N.; Thornalley, P.; Sayed, A.; Fleming, T.; Humpert, P.; Schwenger, V.; Zeier, M.; Hamann, A.; Stern, D.; Brownlee, M.; Bierhaus, A.; Nawroth, P.; Morcos, M. C. elegans as model for the study of high glucose mediated lifespan reduction. Diabetes 58:2450–2456; 2009.
Nordmann, A. J.; Nordmann, A.; Briel, M.; Keller, U.; Yancy Jr., W. S.; Brehm, B. J.; Bucher, H. C. Effects of low-carbohydrate vs low-fat diets on weight loss and cardiovascular risk factors: a meta-analysis of randomized controlled trials. Arch. Intern. Med. 166:285–293; 2006.
Hession, M.; Rolland, C.; Kulkarni, U.; Wise, A.; Broom, J. Systematic review of
randomized controlled trials of low-carbohydrate vs. low-fat/low-calorie diets in the management of obesity and its comorbidities. Obes. Rev. 10:36–50; 2009.
Volek, J. S.; Phinney, S. D.; Forsythe, C. E.; Quann, E. E.; Wood, R. J.; Puglisi, M. J.; Kraemer, W. J.; Bibus, D. M.; Fernandez, M. L.; Feinman, R. D. Carbohydrate restriction has a more favorable impact on the metabolic syndrome than a low fat diet. Lipids 44:297–309; 2009.
332 M. Ristow, S. Schmeisser / Free Radical Biology & Medicine 51 (2011) 327–336
Слайд 7Forsythe, C. E.; Phinney, S. D.; Fernandez, M. L.; Quann, E. E.;
Forsythe, C. E.; Phinney, S. D.; Fernandez, M. L.; Quann, E. E.;
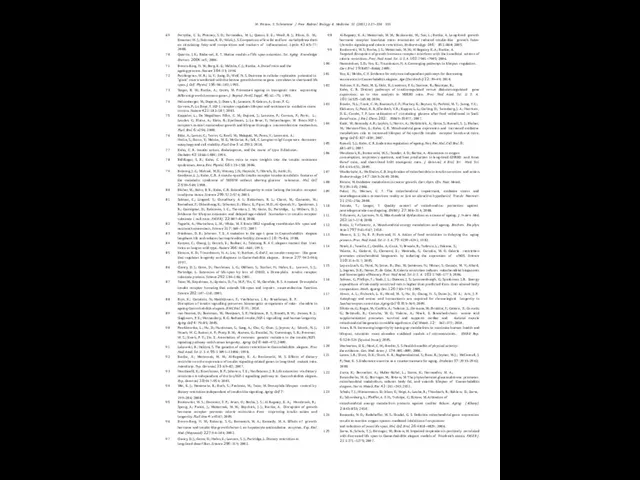
Quarrie, J. K.; Riabowol, K. T. Murine models of life span extension. Sci. Aging Knowledge Environ. 2004:re5; 2004.
Brown-Borg, H. M.; Borg, K. E.; Meliska, C. J.; Bartke, A. Dwarf mice and the
ageing process. Nature 384:33; 1996.
Pendergrass, W. R.; Li, Y.; Jiang, D.; Wolf, N. S. Decrease in cellular replicative potential in "giant" mice transfected with the bovine growth hormone gene correlates to shortened life span. J. Cell. Physiol. 156:96–103; 1993.
Steger, R. W.; Bartke, A.; Cecim, M. Premature ageing in transgenic mice expressing different growth hormone genes. J. Reprod. Fertil. Suppl. 46:61–75; 1993.
Holzenberger, M.; Dupont, J.; Ducos, B.; Leneuve, P.; Geloen, A.; Even, P. C.;
Cervera, P.; Le Bouc, Y. IGF-1 receptor regulates lifespan and resistance to oxidative stress in mice. Nature 421:182–187; 2003.
Kappeler, L.; De Magalhaes Filho, C. M.; Dupont, J.; Leneuve, P.; Cervera, P.; Perin, L.; Loudes, C.; Blaise, A.; Klein, R.; Epelbaum, J.; Le Bouc, Y.; Holzenberger, M. Brain IGF-1 receptors control mammalian growth and lifespan through a neuroendocrine mechanism. PLoS Biol. 6:e254; 2008.
Bitto, A.; Lerner, C.; Torres, C.; Roell, M.; Malaguti, M.; Perez, V.; Lorenzini, A.;
Hrelia, S.; Ikeno, Y.; Matzko, M. E.; McCarter, R.; Sell, C. Long-term Igf-I exposure decreases autophagy and cell viability. PLoS One 5:e12592; 2010.
Kahn, C. R. Insulin action, diabetogenes, and the cause of type II diabetes.
Diabetes 43:1066–1084; 1994.
Biddinger, S. B.; Kahn, C. R. From mice to men: insights into the insulin resistance syndromes. Annu. Rev. Physiol. 68:123–158; 2006.
Brüning, J. C.; Michael, M. D.; Winnay, J. N.; Hayashi, T.; Hörsch, D.; Accili, D.;
Goodyear, L. J.; Kahn, C. R. A muscle-specific insulin receptor knockout exhibits features of the metabolic syndrome of NIDDM without altering glucose tolerance. Mol. Cell 2:559–569; 1998.
Blüher, M.; Kahn, B. B.; Kahn, C. R. Extended longevity in mice lacking the insulin receptor in adipose tissue. Science 299:572–574; 2003.
Selman, C.; Lingard, S.; Choudhury, A. I.; Batterham, R. L.; Claret, M.; Clements, M.; Ramadani, F.; Okkenhaug, K.; Schuster, E.; Blanc, E.; Piper, M. D.; Al-Qassab, H.; Speakman, J. R.; Carmignac, D.; Robinson, I. C.; Thornton, J. M.; Gems, D.; Partridge, L.; Withers, D. J. Evidence for lifespan extension and delayed age-related biomarkers in insulin receptor substrate 1 null mice. FASEB J. 22:807–818; 2008.
Taguchi, A.; Wartschow, L. M.; White, M. F. Brain IRS2 signaling coordinates life span and nutrient homeostasis. Science 317:369–372; 2007.
Friedman, D. B.; Johnson, T. E. A mutation in the age-1 gene in Caenorhabditis elegans lengthens life and reduces hermaphrodite fertility. Genetics 118:75–86; 1988.
Kenyon, C.; Chang, J.; Gensch, E.; Rudner, A.; Tabtiang, R. A C. elegans mutant that lives twice as long as wild type. Nature 366:461–464; 1993.
Kimura, K. D.; Tissenbaum, H. A.; Liu, Y.; Ruvkun, G. daf-2, an insulin receptor- like gene that regulates longevity and diapause in Caenorhabditis elegans. Science 277:942–946; 1997.
Clancy, D. J.; Gems, D.; Harshman, L. G.; Oldham, S.; Stocker, H.; Hafen, E.; Leevers, S. J.; Partridge, L. Extension of life-span by loss of CHICO, a Drosophila insulin receptor substrate protein. Science 292:104–106; 2001.
Tatar, M.; Kopelman, A.; Epstein, D.; Tu, M. P.; Yin, C. M.; Garofalo, R. S. A mutant Drosophila insulin receptor homolog that extends life-span and impairs neuroendocrine function. Science 292:107–110; 2001.
Brys, K.; Castelein, N.; Matthijssens, F.; Vanfleteren, J. R.; Braeckman, B. P.
Disruption of insulin signalling preserves bioenergetic competence of mito- chondria in ageing Caenorhabditis elegans. BMC Biol. 8:91; 2010.
van Heemst, D.; Beekman, M.; Mooijaart, S. P.; Heijmans, B. T.; Brandt, B. W.; Zwaan, B. J.; Slagboom, P. E.; Westendorp, R. G. Reduced insulin/IGF-1 signalling and human longevity. Aging Cell 4:79–85; 2005.
Pawlikowska, L.; Hu, D.; Huntsman, S.; Sung, A.; Chu, C.; Chen, J.; Joyner, A.; Schork, N. J.; Hsueh, W. C.; Reiner, A. P.; Psaty, B. M.; Atzmon, G.; Barzilai, N.; Cummings, S. R.; Browner, W. S.; Kwok, P. Y.; Ziv, E. Association of common genetic variation in the insulin/IGF1 signaling pathway with human longevity. Aging Cell 8:460–472; 2009.
Lakowski, B.; Hekimi, S. The genetics of caloric restriction in Caenorhabditis elegans. Proc. Natl Acad. Sci. U. S. A. 95:13091–13096; 1998.
Bartke, A.; Masternak, M. M.; Al-Regaiey, K. A.; Bonkowski, M. S. Effects of dietary restriction on the expression of insulin-signaling-related genes in long-lived mutant mice. Interdiscip. Top. Gerontol. 35:69–82; 2007.
Houthoofd, K.; Braeckman, B. P.; Johnson, T. E.; Vanfleteren, J. R. Life extension via dietary restriction is independent of the Ins/IGF-1 signalling pathway in Caenorhabditis elegans. Exp. Gerontol. 38:947–954; 2003.
Min, K. J.; Yamamoto, R.; Buch, S.; Pankratz, M.; Tatar, M. Drosophila lifespan control by dietary restriction independent of insulin-like signaling. Aging Cell 7:
199–206; 2008.
Bonkowski, M. S.; Dominici, F. P.; Arum, O.; Rocha, J. S.; Al Regaiey, K. A.; Westbrook, R.; Spong, A.; Panici, J.; Masternak, M. M.; Kopchick, J. J.; Bartke, A. Disruption of growth hormone receptor prevents calorie restriction from improving insulin action and longevity. PLoS One 4:e4567; 2009.
Brown-Borg, H. M.; Rakoczy, S. G.; Romanick, M. A.; Kennedy, M. A. Effects of growth hormone and insulin-like growth factor-1 on hepatocyte antioxidative enzymes. Exp. Biol. Med. (Maywood) 227:94–104; 2002.
Clancy, D. J.; Gems, D.; Hafen, E.; Leevers, S. J.; Partridge, L. Dietary restriction in
long-lived dwarf flies. Science 296:319; 2002.
Al-Regaiey, K. A.; Masternak, M. M.; Bonkowski, M.; Sun, L.; Bartke, A. Long-lived growth hormone receptor knockout mice: interaction of reduced insulin-like growth factor I/insulin signaling and caloric restriction. Endocrinology 146: 851–860; 2005.
Bonkowski, M. S.; Rocha, J. S.; Masternak, M. M.; Al Regaiey, K. A.; Bartke, A.
Targeted disruption of growth hormone receptor interferes with the beneficial actions of calorie restriction. Proc. Natl Acad. Sci. U. S. A. 103:7901–7905; 2006.
Narasimhan, S. D.; Yen, K.; Tissenbaum, H. A. Converging pathways in lifespan regulation. Curr. Biol. 19:R657–R666; 2009.
Yen, K.; Mobbs, C. V. Evidence for only two independent pathways for decreasing senescence in Caenorhabditis elegans. Age (Dordrecht) 32:39–49; 2010.
Yechoor, V. K.; Patti, M. E.; Ueki, K.; Laustsen, P. G.; Saccone, R.; Rauniyar, R.;
Kahn, C. R. Distinct pathways of insulin-regulated versus diabetes-regulated gene expression: an in vivo analysis in MIRKO mice. Proc. Natl Acad. Sci. U. S. A. 101:16525–16530; 2004.
Brooks, N. L.; Trent, C. M.; Raetzsch, C. F.; Flurkey, K.; Boysen, G.; Perfetti, M. T.; Jeong, Y. C.; Klebanov, S.; Patel, K. B.; Khodush, V. R.; Kupper, L. L.; Carling, D.; Swenberg, J. A.; Harrison, D. E.; Combs, T. P. Low utilization of circulating glucose after food withdrawal in Snell dwarf mice. J. Biol. Chem. 282: 35069–35077; 2007.
Katic, M.; Kennedy, A. R.; Leykin, I.; Norris, A.; McGettrick, A.; Gesta, S.; Russell, S. J.; Bluher, M.; Maratos-Flier, E.; Kahn, C. R. Mitochondrial gene expression and increased oxidative metabolism: role in increased lifespan of fat-specific insulin receptor knock-out mice. Aging Cell 6:827–839; 2007.
Russell, S. J.; Kahn, C. R. Endocrine regulation of ageing. Nat. Rev. Mol. Cell Biol. 8:
681–691; 2007.
Westbrook, R.; Bonkowski, M. S.; Strader, A. D.; Bartke, A. Alterations in oxygen
consumption, respiratory quotient, and heat production in long-lived GHRKO and Ames dwarf mice, and short-lived bGH transgenic mice. J. Gerontol. A Biol. Sci. Med. Sci. 64:443–451; 2009.
Wiederkehr, A.; Wollheim, C. B. Implication of mitochondria in insulin secretion and action. Endocrinology 147:2643–2649; 2006.
Ristow, M. Oxidative metabolism in cancer growth. Curr. Opin. Clin. Nutr. Metab.
9:339–345; 2006.
Fukui, H.; Moraes, C. T. The mitochondrial impairment, oxidative stress and neurodegeneration connection: reality or just an attractive hypothesis? Trends Neurosci. 31:251–256; 2008.
Tatsuta, T.; Langer, T. Quality control of mitochondria: protection against neurodegeneration and ageing. EMBO J. 27:306–314; 2008.
Trifunovic, A.; Larsson, N. G. Mitochondrial dysfunction as a cause of ageing. J. Intern. Med. 263:167–178; 2008.
Bratic, I.; Trifunovic, A. Mitochondrial energy metabolism and ageing. Biochim. Biophys. Acta 1797:961–967; 2010.
Masoro, E. J.; Yu, B. P.; Bertrand, H. A. Action of food restriction in delaying the aging process. Proc. Natl Acad. Sci. U. S. A. 79:4239–4241; 1982.
Nisoli, E.; Tonello, C.; Cardile, A.; Cozzi, V.; Bracale, R.; Tedesco, L.; Falcone, S.;
Valerio, A.; Cantoni, O.; Clementi, E.; Moncada, S.; Carruba, M. O. Calorie restriction promotes mitochondrial biogenesis by inducing the expression of eNOS. Science 310:314–317; 2005.
Lopez-Lluch, G.; Hunt, N.; Jones, B.; Zhu, M.; Jamieson, H.; Hilmer, S.; Cascajo, M. V.; Allard, J.; Ingram, D. K.; Navas, P.; de Cabo, R. Calorie restriction induces mitochondrial biogenesis and bioenergetic efficiency. Proc. Natl Acad. Sci. U. S. A. 103:1768–1773; 2006.
Selman, C.; Phillips, T.; Staib, J. L.; Duncan, J. S.; Leeuwenburgh, C.; Speakman, J. R. Energy expenditure of calorically restricted rats is higher than predicted from their altered body composition. Mech. Ageing Dev. 126:783–793; 2005.
Alvers, A. L.; Fishwick, L. K.; Wood, M. S.; Hu, D.; Chung, H. S.; Dunn Jr., W. A.; Aris, J. P. Autophagy and amino acid homeostasis are required for chronological longevity in Saccharomyces cerevisiae. Aging Cell 8:353–369; 2009.
D'Antona, G.; Ragni, M.; Cardile, A.; Tedesco, L.; Dossena, M.; Bruttini, F.; Caliaro, F.; Corsetti, G.; Bottinelli, R.; Carruba, M. O.; Valerio, A.; Nisoli, E. Branched-chain amino acid supplementation promotes survival and supports cardiac and skeletal muscle mitochondrial biogenesis in middle-aged mice. Cell Metab. 12: 362–372; 2010.
Ames, B. N. Increasing longevity by tuning up metabolism: to maximize human health and lifespan, scientists must abandon outdated models of micronutrients. EMBO Rep. 6:S20–S24 (Special Issue); 2005.
Warburton, D. E.; Nicol, C. W.; Bredin, S. S. Health benefits of physical activity:
the evidence. Can. Med. Assoc. J. 174:801–809; 2006.
Lanza, I. R.; Short, D. K.; Short, K. R.; Raghavakaimal, S.; Basu, R.; Joyner, M. J.; McConnell, J. P.; Nair, K. S. Endurance exercise as a countermeasure for aging. Diabetes 57:2933–2942; 2008.
Zarse, K.; Bossecker, A.; Muller-Kuhrt, L.; Siems, K.; Hernandez, M. A.;
Berendsohn, W. G.; Birringer, M.; Ristow, M. The phytochemical glaucarubinone promotes mitochondrial metabolism, reduces body fat, and extends lifespan of Caenorhabditis elegans. Horm. Metab. Res. 43:241–243; 2011.
Schulz, T. J.; Westermann, D.; Isken, F.; Voigt, A.; Laube, B.; Thierbach, R.; Kuhlow, D.; Zarse, K.; Schomburg, L.; Pfeiffer, A. F. H.; Tschöpe, C.; Ristow, M. Activation of
mitochondrial energy metabolism protects against cardiac failure. Aging (Albany) 2:843–853; 2010.
Bonawitz, N. D.; Rodeheffer, M. S.; Shadel, G. S. Defective mitochondrial gene expression results in reactive oxygen species-mediated inhibition of respiration
and reduction of yeast life span. Mol. Cell. Biol. 26:4818–4829; 2006.
Zarse, K.; Schulz, T. J.; Birringer, M.; Ristow, M. Impaired respiration is positively correlated with decreased life span in Caenorhabditis elegans models of Friedreich ataxia. FASEB J. 21:1271–1275; 2007.
M. Ristow, S. Schmeisser / Free Radical Biology & Medicine 51 (2011) 327–336 333
Слайд 8Thierbach, R.; Schulz, T. J.; Isken, F.; Voigt, A.; Mietzner, B.; Drewes,
Thierbach, R.; Schulz, T. J.; Isken, F.; Voigt, A.; Mietzner, B.; Drewes,
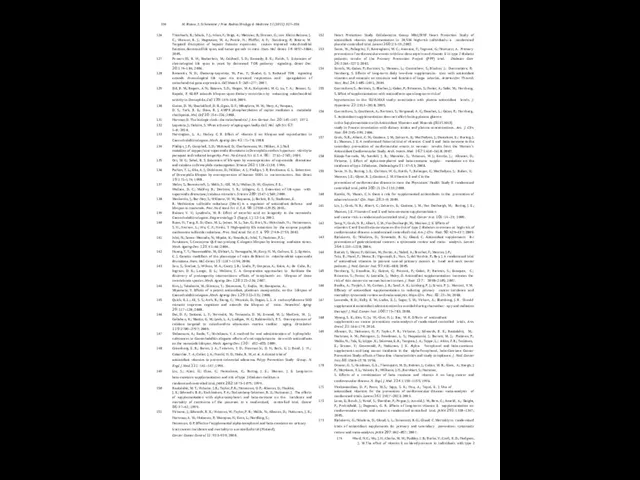
Powers III, R. W.; Kaeberlein, M.; Caldwell, S. D.; Kennedy, B. K.; Fields, S. Extension of chronological life span in yeast by decreased TOR pathway signaling. Genes Dev. 20:174–184; 2006.
Bonawitz, N. D.; Chatenay-Lapointe, M.; Pan, Y.; Shadel, G. S. Reduced TOR signaling extends chronological life span via increased respiration and upregulation of mitochondrial gene expression. Cell Metab. 5:265–277; 2007.
Zid, B. M.; Rogers, A. N.; Katewa, S. D.; Vargas, M. A.; Kolipinski, M. C.; Lu, T. A.; Benzer, S.; Kapahi, P. 4E-BP extends lifespan upon dietary restriction by enhancing mitochondrial activity in Drosophila. Cell 139:149–160; 2009.
Gwinn, D. M.; Shackelford, D. B.; Egan, D. F.; Mihaylova, M. M.; Mery, A.; Vasquez,
D. S.; Turk, B. E.; Shaw, R. J. AMPK phosphorylation of raptor mediates a metabolic checkpoint. Mol. Cell 30:214–226; 2008.
Harman, D. The biologic clock: the mitochondria? J. Am. Geriatr. Soc. 20:145–147; 1972.
Lapointe, J.; Hekimi, S. When a theory of aging ages badly. Cell. Mol. Life Sci. 67:
1–8; 2010.
Harrington, L. A.; Harley, C. B. Effect of vitamin E on lifespan and reproduction in Caenorhabditis elegans. Mech. Ageing Dev. 43:71–78; 1988.
Phillips, J. P.; Campbell, S. D.; Michaud, D.; Charbonneau, M.; Hilliker, A. J. Null
mutation of copper/zinc superoxide dismutase in Drosophila confers hypersen- sitivity to paraquat and reduced longevity. Proc. Natl Acad. Sci. U. S. A. 86: 2761–2765; 1989.
Orr, W. C.; Sohal, R. S. Extension of life-span by overexpression of superoxide dismutase and catalase in Drosophila melanogaster. Science 263:1128–1130; 1994.
Parkes, T. L.; Elia, A. J.; Dickinson, D.; Hilliker, A. J.; Phillips, J. P.; Boulianne, G. L. Extension of Drosophila lifespan by overexpression of human SOD1 in motorneurons. Nat. Genet. 19:171–174; 1998.
Melov, S.; Ravenscroft, J.; Malik, S.; Gill, M. S.; Walker, D. W.; Clayton, P. E.;
Wallace, D. C.; Malfroy, B.; Doctrow, S. R.; Lithgow, G. J. Extension of life-span with superoxide dismutase/catalase mimetics. Science 289:1567–1569; 2000.
Moskovitz, J.; Bar-Noy, S.; Williams, W. M.; Requena, J.; Berlett, B. S.; Stadtman, E.
R. Methionine sulfoxide reductase (MsrA) is a regulator of antioxidant defense and lifespan in mammals. Proc. Natl Acad. Sci. U. S. A. 98:12920–12925; 2001.
Bakaev, V. V.; Lyudmila, M. B. Effect of ascorbic acid on longevity in the nematoda Caenorhabditis elegans. Biogerontology 3 (Suppl. 1):12–16; 2002.
Ruan, H.; Tang, X. D.; Chen, M. L.; Joiner, M. L.; Sun, G.; Brot, N.; Weissbach, H.; Heinemann, S. H.; Iverson, L.; Wu, C. F.; Hoshi, T. High-quality life extension by the enzyme peptide methionine sulfoxide reductase. Proc. Natl Acad. Sci. U. S. A. 99:2748–2753; 2002.
Ishii, N.; Senoo-Matsuda, N.; Miyake, K.; Yasuda, K.; Ishii, T.; Hartman, P. S.;
Furukawa, S. Coenzyme Q10 can prolong C. elegans lifespan by lowering oxidative stress. Mech. Ageing Dev. 125:41–46; 2004.
Huang, T. T.; Naeemuddin, M.; Elchuri, S.; Yamaguchi, M.; Kozy, H. M.; Carlson, E. J.; Epstein, C. J. Genetic modifiers of the phenotype of mice deficient in mitochondrial superoxide dismutase. Hum. Mol. Genet. 15:1187–1194; 2006.
Zou, S.; Sinclair, J.; Wilson, M. A.; Carey, J. R.; Liedo, P.; Oropeza, A.; Kalra, A.; de Cabo, R.; Ingram, D. K.; Longo, D. L.; Wolkow, C. A. Comparative approaches to facilitate the discovery of prolongevity interventions: effects of tocopherols on lifespan of three invertebrate species. Mech. Ageing Dev. 128:222–226; 2007.
Kim, J.; Takahashi, M.; Shimizu, T.; Shirasawa, T.; Kajita, M.; Kanayama, A.;
Miyamoto, Y. Effects of a potent antioxidant, platinum nanoparticle, on the lifespan of Caenorhabditis elegans. Mech. Ageing Dev. 129:322–331; 2008.
Quick, K. L.; Ali, S. S.; Arch, R.; Xiong, C.; Wozniak, D.; Dugan, L. L. A carboxyfullerene SOD mimetic improves cognition and extends the lifespan of mice. Neurobiol. Aging 29:117–128; 2008.
Dai, D. F.; Santana, L. F.; Vermulst, M.; Tomazela, D. M.; Emond, M. J.; MacCoss, M. J.; Gollahon, K.; Martin, G. M.; Loeb, L. A.; Ladiges, W. C.; Rabinovitch, P. S. Overexpression of catalase targeted to mitochondria attenuates murine cardiac aging. Circulation 119:2789–2797; 2009.
Shibamura, A.; Ikeda, T.; Nishikawa, Y. A method for oral administration of hydrophilic substances to Caenorhabditis elegans: effects of oral supplementa- tion with antioxidants on the nematode lifespan. Mech. Ageing Dev. 130: 652–655; 2009.
Greenberg, E. R.; Baron, J. A.; Tosteson, T. D.; Freeman Jr., D. H.; Beck, G. J.; Bond, J. H.; Colacchio, T. A.; Coller, J. A.; Frankl, H. D.; Haile, R. W., et al. A clinical trial of
antioxidant vitamins to prevent colorectal adenoma. Polyp Prevention Study Group. N. Engl. J. Med. 331:141–147; 1994.
Liu, S.; Ajani, U.; Chae, C.; Hennekens, C.; Buring, J. E.; Manson, J. E. Long-term beta-carotene supplementation and risk of type 2 diabetes mellitus: a
randomized controlled trial. JAMA 282:1073–1075; 1999.
Rautalahti, M. T.; Virtamo, J. R.; Taylor, P. R.; Heinonen, O. P.; Albanes, D.; Haukka,
J. K.; Edwards, B. K.; Karkkainen, P. A.; Stolzenberg-Solomon, R. Z.; Huttunen, J. The effects of supplementation with alpha-tocopherol and beta-carotene on the incidence and mortality of carcinoma of the pancreas in a randomized, controlled trial. Cancer 86:37–42; 1999.
Virtamo, J.; Edwards, B. K.; Virtanen, M.; Taylor, P. R.; Malila, N.; Albanes, D.; Huttunen, J. K.; Hartman, A. M.; Hietanen, P.; Maenpaa, H.; Koss, L.; Nordling, S.;
Heinonen, O. P. Effects of supplemental alpha-tocopherol and beta-carotene on urinary tract cancer: incidence and mortality in a controlled trial (Finland).
Cancer Causes Control 11:933–939; 2000.
Heart Protection Study Collaborative Group MRC/BHF Heart Protection Study of antioxidant vitamin supplementation in 20,536 high-risk individuals: a randomised placebo-controlled trial. Lancet 360:23–33; 2002.
Sacco, M.; Pellegrini, F.; Roncaglioni, M. C.; Avanzini, F.; Tognoni, G.; Nicolucci, A. Primary prevention of cardiovascular events with low-dose aspirin and vitamin E in type 2 diabetic patients: results of the Primary Prevention Project (PPP) trial. Diabetes Care 26:3264–3272; 2003.
Zureik, M.; Galan, P.; Bertrais, S.; Mennen, L.; Czernichow, S.; Blacher, J.; Ducimetiere, P.; Hercberg, S. Effects of long-term daily low-dose supplementa- tion with antioxidant vitamins and minerals on structure and function of large arteries. Arterioscler. Thromb. Vasc. Biol. 24:1485–1491; 2004.
Czernichow, S.; Bertrais, S.; Blacher, J.; Galan, P.; Briancon, S.; Favier, A.; Safar, M.; Hercberg, S. Effect of supplementation with antioxidants upon long-term risk of
hypertension in the SU.VI.MAX study: association with plasma antioxidant levels. J. Hypertens. 23:2013–2018; 2005.
Czernichow, S.; Couthouis, A.; Bertrais, S.; Vergnaud, A. C.; Dauchet, L.; Galan, P.; Hercberg, S. Antioxidant supplementation does not affect fasting plasma glucose
in the Supplementation with Antioxidant Vitamins and Minerals (SU.VI.MAX)
study in France: association with dietary intake and plasma concentrations. Am. J. Clin. Nutr. 84:395–399; 2006.
Cook, N. R.; Albert, C. M.; Gaziano, J. M.; Zaharris, E.; MacFadyen, J.; Danielson, E.; Buring, J. E.; Manson, J. E. A randomized factorial trial of vitamins C and E and beta carotene in the secondary prevention of cardiovascular events in women: results from the Women's Antioxidant Cardiovascular Study. Arch. Intern. Med. 167:1610–1618; 2007.
Kataja-Tuomola, M.; Sundell, J. R.; Mannisto, S.; Virtanen, M. J.; Kontto, J.; Albanes, D.; Virtamo, J. Effect of alpha-tocopherol and beta-carotene supple- mentation on the incidence of type 2 diabetes. Diabetologia 51:47–53; 2008.
Sesso, H. D.; Buring, J. E.; Christen, W. G.; Kurth, T.; Belanger, C.; MacFadyen, J.; Bubes, V.; Manson, J. E.; Glynn, R. J.; Gaziano, J. M. Vitamins E and C in the
prevention of cardiovascular disease in men: the Physicians' Health Study II randomized controlled trial. JAMA 300:2123–2133; 2008.
Katsiki, N.; Manes, C. Is there a role for supplemented antioxidants in the prevention of atherosclerosis? Clin. Nutr. 28:3–9; 2009.
Lin, J.; Cook, N. R.; Albert, C.; Zaharris, E.; Gaziano, J. M.; Van Denburgh, M.; Buring, J. E.; Manson, J. E. Vitamins C and E and beta-carotene supplementation
and cancer risk: a randomized controlled trial. J. Natl. Cancer Inst. 101:14–23; 2009.
Song, Y.; Cook, N. R.; Albert, C. M.; Van Denburgh, M.; Manson, J. E. Effects of
vitamins C and E and beta-carotene on the risk of type 2 diabetes in women at high risk of cardiovascular disease: a randomized controlled trial. Am. J. Clin. Nutr. 90:429–437; 2009.
Bjelakovic, G.; Nikolova, D.; Simonetti, R. G.; Gluud, C. Antioxidant supplements for prevention of gastrointestinal cancers: a systematic review and meta- analysis. Lancet 364:1219–1228; 2004.
Bairati, I.; Meyer, F.; Gelinas, M.; Fortin, A.; Nabid, A.; Brochet, F.; Mercier, J. P.;
Tetu, B.; Harel, F.; Masse, B.; Vigneault, E.; Vass, S.; del Vecchio, P.; Roy, J. A randomized trial of antioxidant vitamins to prevent second primary cancers in head and neck cancer patients. J. Natl. Cancer Inst. 97:481–488; 2005.
Hercberg, S.; Ezzedine, K.; Guinot, C.; Preziosi, P.; Galan, P.; Bertrais, S.; Estaquio, C.; Briancon, S.; Favier, A.; Latreille, J.; Malvy, D. Antioxidant supplementation increases the risk of skin cancers in women but not in men. J. Nutr. 137: 2098–2105; 2007.
Bardia, A.; Tleyjeh, I. M.; Cerhan, J. R.; Sood, A. K.; Limburg, P. J.; Erwin, P. J.; Montori, V. M. Efficacy of antioxidant supplementation in reducing primary cancer incidence and mortality: systematic review and meta-analysis. Mayo Clin. Proc. 83:23–34; 2008.
Lawenda, B. D.; Kelly, K. M.; Ladas, E. J.; Sagar, S. M.; Vickers, A.; Blumberg, J. B. Should supplemental antioxidant administration be avoided during chemother- apy and radiation therapy? J. Natl. Cancer Inst. 100:773–783; 2008.
Myung, S. K.; Kim, Y.; Ju, W.; Choi, H. J.; Bae, W. K. Effects of antioxidant
supplements on cancer prevention: meta-analysis of randomized controlled trials. Ann. Oncol. 21:166–179; 2010.
Albanes, D.; Heinonen, O. P.; Taylor, P. R.; Virtamo, J.; Edwards, B. K.; Rautalahti, M.; Hartman, A. M.; Palmgren, J.; Freedman, L. S.; Haapakoski, J.; Barrett, M. J.; Pietinen, P.; Malila, N.; Tala, E.; Liippo, K.; Salomaa, E. R.; Tangrea, J. A.; Teppo, L.; Askin, F. B.; Taskinen, E.; Erozan, Y.; Greenwald, P.; Huttunen, J. K. Alpha- Tocopherol and beta-carotene supplements and lung cancer incidence in the alpha-Tocopherol, beta-Carotene Cancer Prevention Study: effects of base-line characteristics and study compliance. J. Natl. Cancer Inst. 88:1560–1570; 1996.
Omenn, G. S.; Goodman, G. E.; Thornquist, M. D.; Balmes, J.; Cullen, M. R.; Glass, A.; Keogh, J. P.; Meyskens, F. L.; Valanis, B.; Williams, J. H.; Barnhart, S.; Hammar,
S. Effects of a combination of beta carotene and vitamin A on lung cancer and cardiovascular disease. N. Engl. J. Med. 334:1150–1155; 1996.
Vivekananthan, D. P.; Penn, M. S.; Sapp, S. K.; Hsu, A.; Topol, E. J. Use of
antioxidant vitamins for the prevention of cardiovascular disease: meta-analysis of randomised trials. Lancet 361:2017–2023; 2003.
Lonn, E.; Bosch, J.; Yusuf, S.; Sheridan, P.; Pogue, J.; Arnold, J. M.; Ross, C.; Arnold, A.; Sleight, P.; Probstfield, J.; Dagenais, G. R. Effects of long-term vitamin E supplementation on cardiovascular events and cancer: a randomized controlled trial. JAMA 293:1338–1347; 2005.
Bjelakovic, G.; Nikolova, D.; Gluud, L. L.; Simonetti, R. G.; Gluud, C. Mortality in randomized trials of antioxidant supplements for primary and secondary prevention: systematic review and meta-analysis. JAMA 297:842–857; 2007.
Ward, N. C.; Wu, J. H.; Clarke, M. W.; Puddey, I. B.; Burke, V.; Croft, K. D.; Hodgson,
J. M. The effect of vitamin E on blood pressure in individuals with type 2
334 M. Ristow, S. Schmeisser / Free Radical Biology & Medicine 51 (2011) 327–336
Слайд 9diabetes: a randomized, double-blind, placebo-controlled trial. J. Hypertens. 25:
227–234; 2007.
[175] Lippman, S.
diabetes: a randomized, double-blind, placebo-controlled trial. J. Hypertens. 25:
227–234; 2007.
[175] Lippman, S.
![diabetes: a randomized, double-blind, placebo-controlled trial. J. Hypertens. 25: 227–234; 2007. [175]](/_ipx/f_webp&q_80&fit_contain&s_1440x1080/imagesDir/jpg/841510/slide-8.jpg)
G.; Parnes, H. L.; Minasian, L. M.; Gaziano, J. M.; Hartline, J. A.; Parsons, J. K.; Bearden III, J. D.; Crawford, E. D.; Goodman, G. E.; Claudio, J.; Winquist, E.; Cook,
E. D.; Karp, D. D.; Walther, P.; Lieber, M. M.; Kristal, A. R.; Darke, A. K.; Arnold, K. B.; Ganz, P. A.; Santella, R. M.; Albanes, D.; Taylor, P. R.; Probstfield, J. L.; Jagpal, T. J.; Crowley, J. J.; Meyskens Jr., F. L.; Baker, L. H.; Coltman Jr., C. A. Effect of selenium and vitamin E on risk of prostate cancer and other cancers: the Selenium and Vitamin E Cancer Prevention Trial (SELECT). JAMA 301:39–51; 2009.
Barja, G. Oxygen radicals, a failure or a success of evolution? Free Radic. Res. Commun. 18:63–70; 1993.
Rhee, S. G.; Chang, T. S.; Bae, Y. S.; Lee, S. R.; Kang, S. W. Cellular regulation by hydrogen peroxide. J. Am. Soc. Nephrol. 14:S211–S215; 2003.
Kaelin Jr., W. G. ROS: really involved in oxygen sensing. Cell Metab. 1:357–358; 2005.
Connor, K. M.; Subbaram, S.; Regan, K. J.; Nelson, K. K.; Mazurkiewicz, J. E.; Bartholomew, P. J.; Aplin, A. E.; Tai, Y. T.; Aguirre-Ghiso, J.; Flores, S. C.; Melendez,
J. A. Mitochondrial H2O2 regulates the angiogenic phenotype via PTEN oxidation.
J. Biol. Chem. 280:16916–16924; 2005.
Guzy, R. D.; Hoyos, B.; Robin, E.; Chen, H.; Liu, L.; Mansfield, K. D.; Simon, M. C.;
Hammerling, U.; Schumacker, P. T. Mitochondrial complex III is required for hypoxia-induced ROS production and cellular oxygen sensing. Cell Metab. 1: 401–408; 2005.
Guzy, R. D.; Schumacker, P. T. Oxygen sensing by mitochondria at complex III: the paradox of increased reactive oxygen species during hypoxia. Exp. Physiol. 91:807–819; 2006.
Chandel, N. S.; Budinger, G. R. The cellular basis for diverse responses to oxygen.
Free Radic. Biol. Med. 42:165–174; 2007.
Veal, E. A.; Day, A. M.; Morgan, B. A. Hydrogen peroxide sensing and signaling.
Mol. Cell 26:1–14; 2007.
Owusu-Ansah, E.; Yavari, A.; Mandal, S.; Banerjee, U. Distinct mitochondrial retrograde signals control the G1–S cell cycle checkpoint. Nat. Genet. 40: 356–361; 2008.
Finley, L. W.; Haigis, M. C. The coordination of nuclear and mitochondrial communication during aging and calorie restriction. Ageing Res. Rev. 8:173–188; 2009.
Ristow, M.; Zarse, K.; Oberbach, A.; Klöting, N.; Birringer, M.; Kiehntopf, M.;
Stumvoll, M.; Kahn, C. R.; Blüher, M. Antioxidants prevent health-promoting effects of physical exercise in humans. Proc. Natl Acad. Sci. U. S. A. 106: 8665–8670; 2009.
Loh, K.; Deng, H.; Fukushima, A.; Cai, X.; Boivin, B.; Galic, S.; Bruce, C.; Shields, B. J.; Skiba, B.; Ooms, L. M.; Stepto, N.; Wu, B.; Mitchell, C. A.; Tonks, N. K.; Watt, M. J.; Febbraio, M. A.; Crack, P. J.; Andrikopoulos, S.; Tiganis, T. Reactive oxygen species enhance insulin sensitivity. Cell Metab. 10:260–272; 2009.
Lee, S. J.; Hwang, A. B.; Kenyon, C. Inhibition of respiration extends C. elegans life span via reactive oxygen species that increase HIF-1 activity. Curr. Biol. 20: 2131–2136; 2010.
Yang, W.; Hekimi, S. A Mitochondrial superoxide signal triggers increased longevity in Caenorhabditis elegans. PLoS Biol. 8:e1000556; 2010.
Woo, D. K.; Shadel, G. S. Mitochondrial stress signals revise an old aging theory.
Cell 144:11–12; 2011.
Boveris, A.; Chance, B. The mitochondrial generation of hydrogen peroxide: general properties and effect of hyperbaric oxygen. Biochem. J. 134:707–716; 1973.
Cypser, J. R.; Johnson, T. E. Multiple stressors in Caenorhabditis elegans induce stress hormesis and extended longevity. J. Gerontol. A Biol. Sci. Med. Sci. 57: B109–B114; 2002.
Yanase, S.; Hartman, P. S.; Ito, A.; Ishii, N. Oxidative stress pretreatment increases the X-radiation resistance of the nematode Caenorhabditis elegans. Mutat. Res. 426:31–39; 1999.
Turrens, J. F. Mitochondrial formation of reactive oxygen species. J. Physiol. 552:
335–344; 2003.
Conti, B.; Sanchez-Alavez, M.; Winsky-Sommerer, R.; Morale, M. C.; Lucero, J.; Brownell, S.; Fabre, V.; Huitron-Resendiz, S.; Henriksen, S.; Zorrilla, E. P.; de
Lecea, L.; Bartfai, T. Transgenic mice with a reduced core body temperature have an increased life span. Science 314:825–828; 2006.
Hosono, R.; Mitsui, Y.; Sato, Y.; Aizawa, S.; Miwa, J. Life span of the wild and mutant nematode Caenorhabditis elegans: effects of sex, sterilization, and
temperature. Exp. Gerontol. 17:163–172; 1982.
Ali, S. S.; Marcondes, M. C.; Bajova, H.; Dugan, L. L.; Conti, B. Metabolic depression and increased ROS production by isolated mitochondria at moderately lower temperatures. J. Biol. Chem. 285:32522–32528; 2010.
Masoro, E. J. Influence of caloric intake on aging and on the response to stressors.
J. Toxicol. Environ. Health B Crit. Rev. 1:243–257; 1998.
Yu, B. P.; Chung, H. Y. Stress resistance by caloric restriction for longevity. Ann. N. Y. Acad. Sci. 928:39–47; 2001.
Masoro, E. J. The role of hormesis in life extension by dietary restriction.
Interdiscip. Top. Gerontol. 35:1–17; 2007.
Southam, C. M.; Ehrlich, J. Effects of extract of western red-cedar heartwood on certain wood-decaying fungi in culture. Phytopathology 33:517–524; 1943.
Calabrese, E. J.; Baldwin, L. A. Defining hormesis. Hum. Exp. Toxicol. 21:91–97; 2002.
Cypser, J. R.; Tedesco, P.; Johnson, T. E. Hormesis and aging in Caenorhabditis elegans. Exp. Gerontol. 41:935–939; 2006.
Rattan, S. I. Hormesis in aging. Ageing Res. Rev. 7:63–78; 2008.
Mattson, M. P. Hormesis defined. Ageing Res. Rev. 7:1–7; 2008.
Tapia, P. C. Sublethal mitochondrial stress with an attendant stoichiometric
augmentation of reactive oxygen species may precipitate many of the beneficial alterations in cellular physiology produced by caloric restriction, intermittent fasting, exercise and dietary phytonutrients: 'mitohormesis' for health and vitality. Med. Hypotheses 66:832–843; 2006.
Sreekumar, R.; Unnikrishnan, J.; Fu, A.; Nygren, J.; Short, K. R.; Schimke, J.; Barazzoni, R.; Nair, K. S. Effects of caloric restriction on mitochondrial function and gene transcripts in rat muscle. Am. J. Physiol. Endocrinol. Metab. 283: E38–E43; 2002.
Kharade, S. V.; Mittal, N.; Das, S. P.; Sinha, P.; Roy, N. Mrg19 depletion increases
S. cerevisiae lifespan by augmenting ROS defence. FEBS Lett. 579:6809–6813; 2005.
Piper, P. W.; Harris, N. L.; MacLean, M. Preadaptation to efficient respiratory
maintenance is essential both for maximal longevity and the retention of replicative potential in chronologically ageing yeast. Mech. Ageing Dev. 127: 733–740; 2006.
Huang, T. T.; Carlson, E. J.; Gillespie, A. M.; Shi, Y.; Epstein, C. J. Ubiquitous overexpression of CuZn superoxide dismutase does not extend life span in mice.
J. Gerontol. A Biol. Sci. Med. Sci. 55:B5–B9; 2000.
Bayne, A. C.; Sohal, R. S. Effects of superoxide dismutase/catalase mimetics on life
span and oxidative stress resistance in the housefly, Musca domestica. Free Radic. Biol. Med. 32:1229–1234; 2002.
Keaney, M.; Gems, D. No increase in lifespan in Caenorhabditis elegans upon treatment with the superoxide dismutase mimetic EUK-8. Free Radic. Biol. Med. 34:277–282; 2003.
Andziak, B.; O'Connor, T. P.; Qi, W.; DeWaal, E. M.; Pierce, A.; Chaudhuri, A. R.; Van Remmen, H.; Buffenstein, R. High oxidative damage levels in the longest- living rodent, the naked mole-rat. Aging Cell 5:463–471; 2006.
Selman, C.; McLaren, J. S.; Meyer, C.; Duncan, J. S.; Redman, P.; Collins, A. R.; Duthie, G. G.; Speakman, J. R. Life-long vitamin C supplementation in combination with cold exposure does not affect oxidative damage or lifespan in mice, but decreases expression of antioxidant protection genes. Mech. Ageing Dev. 127:897–904; 2006.
Ran, Q.; Liang, H.; Ikeno, Y.; Qi, W.; Prolla, T. A.; Roberts II, L. J.; Wolf, N.; Van Remmen, H.; Richardson, A. Reduction in glutathione peroxidase 4 increases life span through increased sensitivity to apoptosis. J. Gerontol. A Biol. Sci. Med. Sci.
62:932–942; 2007.
Doonan, R.; McElwee, J. J.; Matthijssens, F.; Walker, G. A.; Houthoofd, K.; Back, P.; Matscheski, A.; Vanfleteren, J. R.; Gems, D. Against the oxidative damage theory of aging: superoxide dismutases protect against oxidative stress but have little or no effect on life span in Caenorhabditis elegans. Genes Dev. 22:3236–3241; 2008.
Heidler, T.; Hartwig, K.; Daniel, H.; Wenzel, U. Caenorhabditis elegans lifespan extension caused by treatment with an orally active ROS-generator is dependent on DAF-16 and SIR-2.1. Biogerontology 11:183–195; 2010.
Jang, Y. C.; van Remmen, H. The mitochondrial theory of aging: insight from transgenic and knockout mouse models. Exp. Gerontol. 44:256–260; 2009.
Jang, Y. C.; Perez, V. I.; Song, W.; Lustgarten, M. S.; Salmon, A. B.; Mele, J.; Qi, W.; Liu, Y.; Liang, H.; Chaudhuri, A.; Ikeno, Y.; Epstein, C. J.; Van Remmen, H.;
Richardson, A. Overexpression of Mn superoxide dismutase does not increase life span in mice. J. Gerontol. A Biol. Sci. Med. Sci. 64:1114–1125; 2009.
Lapointe, J.; Stepanyan, Z.; Bigras, E.; Hekimi, S. Reversal of the mitochondrial
phenotype and slow development of oxidative biomarkers of aging in long-lived Mclk1+/− mice. J. Biol. Chem. 284:20364–20374; 2009.
Van Raamsdonk, J. M.; Hekimi, S. Deletion of the mitochondrial superoxide dismutase sod-2 extends lifespan in Caenorhabditis elegans. PLoS Genet. 5: e1000361; 2009.
Yen, K.; Patel, H. B.; Lublin, A. L.; Mobbs, C. V. SOD isoforms play no role in lifespan in ad lib or dietary restricted conditions, but mutational inactivation of SOD-1 reduces life extension by cold. Mech. Ageing Dev. 130:173–178; 2009.
Zhang, Y.; Ikeno, Y.; Qi, W.; Chaudhuri, A.; Li, Y.; Bokov, A.; Thorpe, S. R.; Baynes,
J. W.; Epstein, C.; Richardson, A.; Van Remmen, H. Mice deficient in both Mn superoxide dismutase and glutathione peroxidase-1 have increased oxidative damage and a greater incidence of pathology but no reduction in longevity. J. Gerontol. A Biol. Sci. Med. Sci. 64:1212–1220; 2009.
Pun, P. B.; Gruber, J.; Tang, S. Y.; Schaffer, S.; Ong, R. L.; Fong, S.; Ng, L. F.; Cheah, I.;
Halliwell, B. Ageing in nematodes: do antioxidants extend lifespan in Caenorhabditis elegans? Biogerontology 11:17–30; 2010.
Sanz, A.; Fernandez-Ayala, D. J.; Stefanatos, R. K.; Jacobs, H. T. Mitochondrial ROS production correlates with, but does not directly regulate lifespan in Drosophila. Aging (Albany) 2:200–203; 2010.
Zintel, S.; Schwitalla, D.; Luce, K.; Hamann, A.; Osiewacz, H. D. Increasing mitochondrial superoxide dismutase abundance leads to impairments in protein quality control and ROS scavenging systems and to lifespan shortening. Exp. Gerontol. 45:525–532; 2010.
Lam, Y. T.; Stocker, R.; Dawes, I. W. The lipophilic antioxidants α-tocopherol and coenzyme Q10 reduce the replicative lifespan of Saccharomyces cerevisiae. Free Radic. Biol. Med. 49:237–244; 2010.
Lithgow, G. J.; White, T. M.; Melov, S.; Johnson, T. E. Thermotolerance and extended life-span conferred by single-gene mutations and induced by thermal stress. Proc. Natl Acad. Sci. U. S. A. 92:7540–7544; 1995.
Vanfleteren, J. R.; De Vreese, A. The gerontogenes age-1 and daf-2 determine metabolic rate potential in aging Caenorhabditis elegans. FASEB J. 9:1355–1361; 1995.
M. Ristow, S. Schmeisser / Free Radical Biology & Medicine 51 (2011) 327–336 335